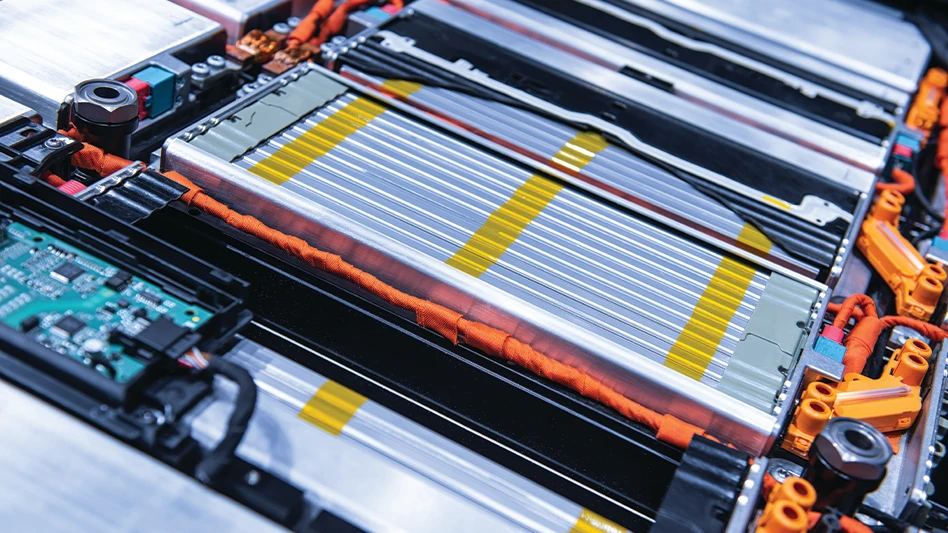
Sergii | stock.adobe.com
The shift of electric vehicles (EVs) from a niche market to a central component of the automotive industry has had significant impacts and promises to bring many more.
As the scale of lithium-ion battery (LIB) use expands, concerns about the potential scarcity of essential metals and the environmental damage from mineral extraction have grown. This highlights the urgent need for effective battery recycling.
Large-scale production of LIBs has created a virtuous cycle: economies of scale drive cost reductions, making widespread adoption more feasible and encouraging further expansion. As a result, substantial quantities of valuable materials such as lithium, cobalt and copper soon will be found in used batteries on our streets and in our garages.
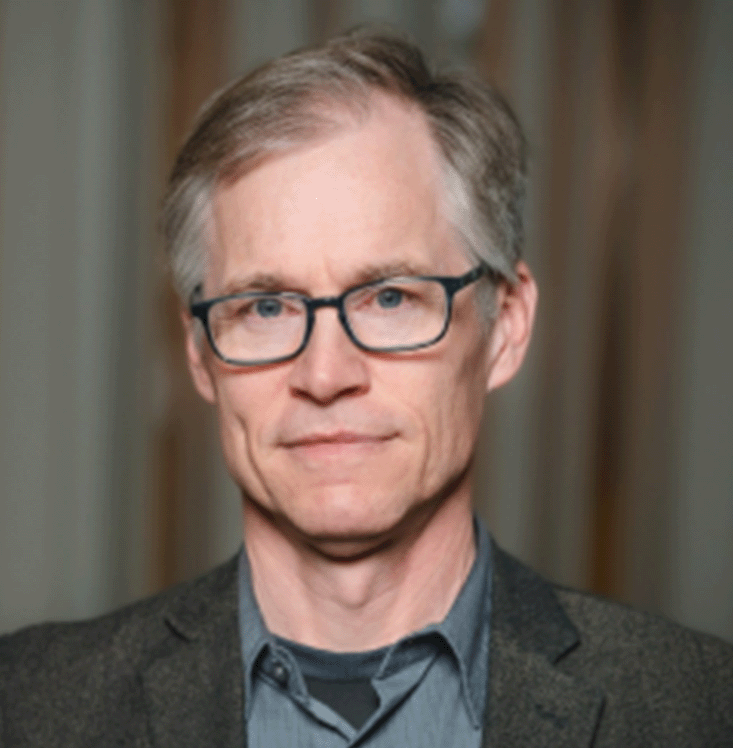
Recognizing this, Europe and China have heavily invested in battery recycling, with China leading the world in recycling capacity. In the U.S., Redwood Materials, founded by former Tesla Chief Technology Officer J.B. Straubel, is a pioneering force in industrial-scale battery recycling, having attracted nearly $2 billion in investment and forming partnerships with major automotive and battery companies. Battery recycling already has become a global industry, and its importance will continue to grow.
Let’s explore the global context of battery raw materials, different recycling approaches, and how emerging battery chemistries could impact recycling efforts today and into the future.
EV batteries in the global context
The scale of electrification with batteries has elevated it to a matter of national security and economic competitiveness for many countries.
The battery supply chain is extensive, starting with raw material mining and ending with battery cell manufacturing. The raw materials needed for batteries are unevenly distributed globally. South American countries—especially Bolivia, Argentina and Chile—hold the largest lithium reserves, followed by the U.S., Australia and China. China dominates both ends of the supply chain, controlling a significant portion of battery manufacturing (79 percent in 2021) with additional contracts or control over major reserves outside its borders.
China’s strong position in lithium supply has prompted the exploration of alternative extraction methods, such as direct lithium extraction, which is being commercialized by existing petroleum companies. In response to a limited domestic battery manufacturing base, the U.S. has implemented initiatives such as the Inflation Reduction Act, aimed at boosting investment in battery materials, manufacturing and recycling. Legislative efforts to strengthen the battery supply chain are expected to continue across the US. Similar strategies in Europe and China have contributed to their advanced capabilities in the sector.
This demonstrates how the global competition for critical battery materials is an established obstacle that is expected to persist. However, many nations already have integrated this into their long-term strategic planning, securing access to resources essential to battery manufacturing while optimizing the use of existing materials. A number of approaches are being tested or employed to stabilize the battery supply chain and safeguard access to critical natural resources.
Reuse
One solution for addressing this issue is the process of reuse. Though not typically considered recycling, reuse is an established method for maximizing resource utilization. In fact, reuse is often preferred because it requires minimal processing compared to breaking down materials for reconstruction.
In the context of LIBs, repurposing used automotive batteries for stationary power storage can complement clean energy sources like solar or wind. This practice is both viable and beneficial as it allows additional value to be obtained from what otherwise would be considered a spent resource and provides a low-cost option to those exploring energy storage projects.
A significant “gray market” exists, utilizing used EV batteries in do-it-yourself (DIY) power storage. A used 50-kilowatt (kW) battery pack from a small EV can hold more than enough power to effectively serve as backup power for a home. As the number of used EV batteries increases, repurposing them for stationary storage likely will become more common.
Battery capacity and life span
Battery capacity degrades with repeated use (see figure below). We commonly experience this when using cell phones over an extended period, and this same principle holds true in electric vehicles.
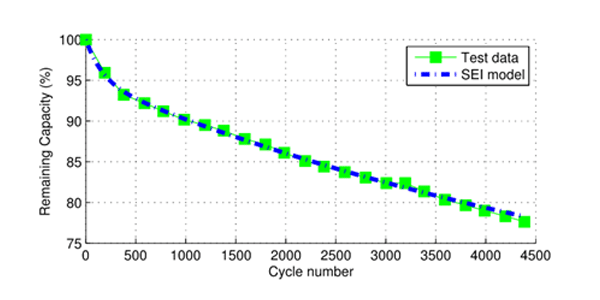
Strategies exist to maximize battery life span, but many batteries often outlive the devices they power. For example, 3,500 cycles would be more than nine years of cycling the battery every day. In EVs, a battery is considered obsolete when its capacity drops to 80 percent of its original specification. However, a battery that originally had a 100-kWh capacity still holds 80 kWh, enough to power most homes for several days. Thus, a spent EV battery can have a second life in stationary applications.
Recycling battery materials
When a battery reaches the end of its useful life, it must be broken down to recover its components for reuse in new batteries. This “true” recycling involves more complex processes compared to the reuse of EV batteries. All methods involve considerable disassembly and/or destruction of the battery packs, modules and cells and can be broadly classified into three categories:
● Mechanical recycling: This method involves disassembling the battery pack and physically recovering the active materials for reuse or recycling with relatively low processing energy. It requires more up-front labor but yields high-purity materials and is suitable for battery packs that allow for easy disassembly. However, as EV designers optimize battery density, moving from cell-module packs to cell-pack architectures, a manufacturing process used to create LIB packs by directly integrating individual battery cells into the pack without using module-level electronics, they could design out modular components, potentially making mechanical recycling more challenging.
● Pyrometallurgical recycling: This process uses heat to burn off nonmetallic components, leaving behind a high-grade ore. The resulting material is refined to recover valuable metals. While applicable to various battery configurations, this method requires specialized equipment and subsequent mineral refining and generates high energy costs and waste gases.
● Hydrometallurgical recycling: This approach utilizes mechanical destruction followed by the extraction of valuable components through liquid leaching. It can be used on nearly any battery configuration and recovers metals with high purity. The downside of this method is that it entails additional processing for recovery and generates a wastewater stream that requires treatment.
Each method has its benefits and drawbacks, with hydrometallurgical recycling being the most widely utilized method across the industry. While they do not require entirely new chemistry, they demand significant investment in equipment and infrastructure. However, these practices are important as they will provide more raw material supply security, encouraging further development while helping to keep battery prices on a downward trend.
The supply of used EV batteries today is fairly low but will increase steadily with time. This ramp-up period should provide time to refine and optimize industrial practices, creating a strong foundation for expansion as more batteries reach end-of-life.
Changes in LIB chemistry
Addressing raw material issues also can be achieved by modifying battery chemistry.
Sponsored Content
Still relying on manual sorters?
Let AI do the heavy lifting. Waste Robotics delivers reliable, high-performance robots tailored for complex waste streams. They require minimal maintenance, are easy to operate, and are designed to boost your recovery rates. Smarter sorting starts with the right partner. Waste Expo Booth #1969 & REMA #2843
Click here to see our robots in action!Sponsored Content
Still relying on manual sorters?
Let AI do the heavy lifting. Waste Robotics delivers reliable, high-performance robots tailored for complex waste streams. They require minimal maintenance, are easy to operate, and are designed to boost your recovery rates. Smarter sorting starts with the right partner. Waste Expo Booth #1969 & REMA #2843
Click here to see our robots in action!Sponsored Content
Still relying on manual sorters?
Let AI do the heavy lifting. Waste Robotics delivers reliable, high-performance robots tailored for complex waste streams. They require minimal maintenance, are easy to operate, and are designed to boost your recovery rates. Smarter sorting starts with the right partner. Waste Expo Booth #1969 & REMA #2843
Click here to see our robots in action!Sponsored Content
Still relying on manual sorters?
Let AI do the heavy lifting. Waste Robotics delivers reliable, high-performance robots tailored for complex waste streams. They require minimal maintenance, are easy to operate, and are designed to boost your recovery rates. Smarter sorting starts with the right partner. Waste Expo Booth #1969 & REMA #2843
Click here to see our robots in action!Sponsored Content
Still relying on manual sorters?
Let AI do the heavy lifting. Waste Robotics delivers reliable, high-performance robots tailored for complex waste streams. They require minimal maintenance, are easy to operate, and are designed to boost your recovery rates. Smarter sorting starts with the right partner. Waste Expo Booth #1969 & REMA #2843
Click here to see our robots in action!Sponsored Content
Still relying on manual sorters?
Let AI do the heavy lifting. Waste Robotics delivers reliable, high-performance robots tailored for complex waste streams. They require minimal maintenance, are easy to operate, and are designed to boost your recovery rates. Smarter sorting starts with the right partner. Waste Expo Booth #1969 & REMA #2843
Click here to see our robots in action!Sponsored Content
Still relying on manual sorters?
Let AI do the heavy lifting. Waste Robotics delivers reliable, high-performance robots tailored for complex waste streams. They require minimal maintenance, are easy to operate, and are designed to boost your recovery rates. Smarter sorting starts with the right partner. Waste Expo Booth #1969 & REMA #2843
Click here to see our robots in action!Sponsored Content
Still relying on manual sorters?
Let AI do the heavy lifting. Waste Robotics delivers reliable, high-performance robots tailored for complex waste streams. They require minimal maintenance, are easy to operate, and are designed to boost your recovery rates. Smarter sorting starts with the right partner. Waste Expo Booth #1969 & REMA #2843
Click here to see our robots in action!The potential for revolutionary new battery technologies has received significant press as the quest for more energy density has the potential to supercharge the EV industry. Although these innovations could emerge, current approaches offer promising solutions to reduce material supply concerns.
For example, nickel manganese cobalt (NMC) batteries at a 1:1:1 ratio, which have been widely used, are shifting towards lower proportions of cobalt and manganese to reduce costs. Lithium iron phosphate (LFP) batteries, once considered less suitable for EVs due to lower specific energy, are gaining traction due to advances in packaging and chemistry. LFP batteries now are a popular choice for both EVs and stationary applications. Though they still require lithium, these batteries reduce the reliance on nickel, manganese and cobalt, enabling them to penetrate markets previously dominated by NMC.
Recycling’s important role
In the rapidly evolving world of EV batteries, recycling is becoming increasingly crucial and is already being implemented on an industrial scale. Driven by concerns over raw material supply, geopolitical competition, and the need for more efficient resource management, recycling will clearly play a significant role in the EV battery supply chain of the future.
As we are still in the early stages of technology development for EVs and their batteries, there likely will be challenges ahead. With these challenges will come opportunities for innovation that should drive sustainability efforts across the entire EV life cycle.
The automotive industry can expect to see recycling technology improve, alleviating the threat of raw material constraints and scarcity. The valuable resources that drive EV performance will, in turn, continue to be available for future generations, powering transportation while promoting a greener future.
Battery performance metrics key
Understanding battery performance involves several key metrics (see table below), including specific energy, specific power, safety, life span and cost. Optimizing one metric often has implications on others, so understanding the characteristics of the various battery chemistries, along with the needs of each application, is critical. The specific energy of a stationary battery in a backup power unit is not particularly important. But in a mobile application, specific energy is paramount. This could make different battery chemistries preferred in different settings and could lead to challenges in recycling as a more diverse stream of battery types must be accommodated.
Performance Dimension | Units | Description |
Specific Energy | W-h/kg | A measure of the energy capacity relative to a battery’s weight |
Specific Power | C (Coulomb) | The ability to deliver current, which determines the amount of work the battery can support |
Safety | °C | The temperature above which the battery becomes unstable and uncontrolled chemical reactions (fire) are likely to occur |
Performance | Multiple | Includes considerations such as changes in storage capacity with temperature, self-discharge rate, and differences in charging or discharging rates supported |
Life span | Charge-discharge cycles | A measure of the useful operational lifetime of the battery usually expressed as a range due to the variable effects of temperature, charge/discharge depth, rate, etc. |
Cost | $/kWh | Used to allow cost comparisons |
Timothy Gotsick is the vice president of technology and innovation at MacDermid Enthone Industrial Solutions, a Waterbury, Connecticut-based manufacturer of chemical compounds catering to all facets of surface finishing applications. To learn more, visit www.macdermidenthone.com.
Get curated news on YOUR industry.
Enter your email to receive our newsletters.
Latest from Recycling Today
- Massive Chinese steelmaking rebound recorded in March
- LME looks into sustainable metal pricing
- OnePlanet Solar Recycling closes $7M seed financing round
- AMCS launches AMCS Platform Spring 2025 update
- Cyclic Materials to build rare earth recycling facility in Mesa, Arizona
- Ecobat’s Seculene product earns recognition for flame-retardant properties
- IWS’ newest MRF is part of its broader strategy to modernize waste management infrastructure
- PCA reports profitable Q1