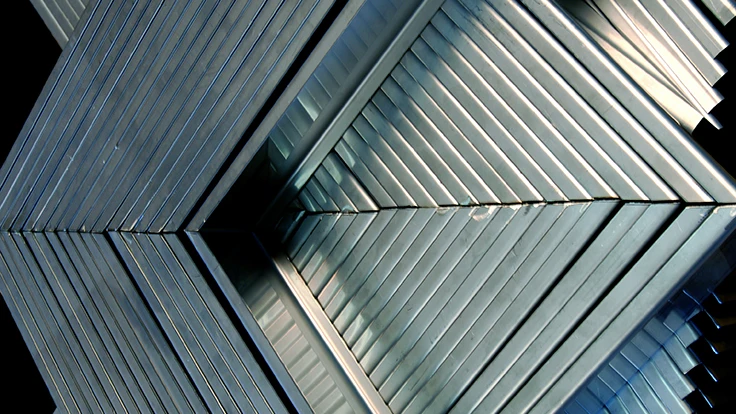
[The following article is an excerpt from “Aluminium Recyclability and Recycling: Towards Sustainable Cities,” written by Michael Stacey and published by Cwningen Press in 2015. The document can be read in its entirety here.]
The advantages of recycling aluminium include:
- significant energy savings of up to 95% compared to the production of primary aluminium;
- reduced waste disposal and landfill; and
- reduced capital cost of only 10% compared to primary smelting.
Recycling aluminium requires up to 95% less primary energy than producing aluminium from bauxite. Within Europe, the primary energy required to produce primary aluminium ingots is 157 megajoules (MJ) per kilogram (kg). (However, this figure is dependent on the efficiency of the energy mix used for smelting). In comparison, the primary energy required to produce recycled aluminium ingots is 7.85 MJ per kg. This is based on a 50/50 aluminium mix of process scrap and post-consumer scrap, such as recycled window frames.
Embodied Energy in the Building and Construction Industry
Embodied energy is “the initial energy investment required to produce a material or product.” It includes the energy required for extraction of natural resources, manufacturing, transportation and construction, but critically it does not include use phase or end-of-life energy savings and therefore cannot be used for comparative purposes.
Therefore, the total embodied energy of a building or building material is the total amount of energy necessary to produce and process all materials (from virgin or recycled sources) required to bring a product or full building into operation. In the 1970s and 1980s, there was a movement to calculate the embodied energy of buildings and building products as a way of quantifying the value of construction, renovation and reuse of building components and complete structures. At the time, the argument made was that saving buildings from demolition, and therefore keeping that embodied energy locked up in existing building stock, could be equated with energy savings and support a broader sustainability agenda.
In economic terms, embodied energy locked up in existing building stock can be seen as a “sunk cost,” posing no inherent energy saving since that energy expenditure occurred in the past, as did the environmental impacts already incurred. In this paradigm, the only real saving associated with retaining existing building stock, or building components, is the avoidance of environmental impacts associated with not constructing or manufacturing the new building or product.
Three variables in particular inform the embodied energy approach for evaluating aluminium in building components:
- Aluminium has a very high recycling rate and it retains its value in terms of embodied energy through recovery, reprocessing and remanufacture, resulting in a large difference between the embodied energy of primary and recycled aluminium. For this reason, the allocation of impacts from raw material production must be equitably (and consistently) shared between products through careful end-of-life modelling, a practice that is well developed but constantly evolving.
- Aluminium’s exceptional durability challenges the direct comparison of products with different base materials, such as aluminium, steel, wood and plastic mullions, in terms of initial material inputs, as building products may have large differences in expected usable life and therefore different replacement cycles over the lifetime of a building.
- Newly manufactured building components often display higher efficiency related to savings in operational energy – a crucial component of understanding the full impacts of building and construction. Typically, this is influenced by the other materials in an aluminium-based building assembly, such as the insulating core of an aluminium composite panel or a double- or triple-glazed unit within a high-performance aluminium curtain-walling system.
Together, these three factors make studies of embodied energy that rely on cradle-to-gate life cycle analysis (LCA) of materials or typical assemblies less useful for aluminium than they may be for more basic building materials such as brick and concrete block. As many of the unique attributes of aluminium are evidenced over time, it is no surprise that assessments with limited scope are unable to fully characterise these attributes and may be less able to accurately compare products and assemblies across material types.
A more holistic building life cycle approach to embodied energy accounting places less emphasis on the product at the point of sale and allows for a comparison of materials and products based on their intended performance and their role in architectural assemblies and building systems.
This approach allows designers and building owners to ask questions that are directly tied to design and management decisions, exploring relationships such as the comparison of the value of retaining building components with the production of newer, potentially more efficient assemblies. It can also productively inform other aspects of the sustainability conversation, such as material durability, as shown in the chapter “Life Cycle Assessment of Window-Framing Materials in Aluminium” and in “Life Cycle Thinking,” the third report of the Towards Sustainable Cities Research Programme.
Embodied Energy of Aluminium
Geoffrey Hammond, Craig Jones, Fiona Lowrie and Peter Tse provide a more complete definition of embodied energy in “Embodied Carbon: The Inventory of Carbon and Energy [ICE].” This inventory includes both embodied energy and embodied carbon.
Embodied energy [is] the total primary energy consumed [from] direct and indirect processes associated with a product or service and within the boundaries of cradle-to-gate. This includes all activities from material extraction (quarrying/mining), manufacturing, transportation and right through to fabrication processes until the product is ready to leave the final factory gate.
Caution needs to be exercised in the use of embodied energy figures, as often the published figures are based on historic data and therefore do not allow for improvements in production techniques and reductions in energy requirements, as noted above. Hammond and Jones also observe:
“There is a considerable temptation for inexperienced users of the ICE database to examine the embodied energy and carbon values as printed in the summary tables to instantly ‘determine’ the ‘best’ materials. This type of analysis must not be completed. The data within the ICE database is typically in the units MJ or kg of CO2 per kg of material, which is not a fair functional unit for material comparisons.
They cite aluminium as a case for a more informed approach:
“An example of a set function is the examination of wall cladding systems. There are competing cladding systems and materials and each set will have different material quantity requirements. For example, aluminium has a lower density than steel and so aluminium cladding would typically require a smaller mass of material than if the cladding were made from steel.”
Using the end-of-life recycling method, ICE states that the embodied energy of extruded aluminium is 53 MJ/kg, with an embodied carbon figure of 3 kgCO2e/kg. ICE is specifically focused on the United Kingdom and the figures are based on a recycling rate of 90% and a process loss of 2%. This recycling rate for aluminium is somewhat pessimistic given that a TU Delft study recorded rates of 92% to 98%, with the recycling rate from the aluminium roof and other components of Wembley Stadium recorded as 96%.
The Importance of Embodied Energy in Low-Energy Architecture
With the successful delivery of low-energy architecture, the embodied energy of building materials and construction becomes increasingly important. “The energy consumption attributed to a building material’s life cycle may at present be comparatively far less than operations energy, with current figures indicating that building materials represent anywhere from 2 to 38 per cent of a building’s lifetime energy consumption depending upon building type and location. However, this range has increased from 9 to 46 per cent for high performance buildings as a result of voluntary standards and stringent energy codes within building regulations.”
Full life cycle impact assessment is likely to grow in importance and popularity in the near future as low-carbon energy-efficient buildings become the norm and programmes such as the Architecture 2030 Challenge for Products, BREEAM (Building Research Establishment Environmental Assessment Method) and LEED (Leadership in Energy and Environmental Design) continue to advocate life cycle modelling for architecture products. This is a component of a design approach that seeks to decrease the carbon footprint of projects, progressing towards carbon-neutral buildings.
From Embodied Energy to Environmental Impacts
In the last decade, as the methods and means of environmental assessment have evolved, there has been a move away from a reliance on proxy measures of sustainability, such as embodied energy, and towards direct measures of environmental impacts (including global warming, acidification, eutrophication, photochemical smog formation, ozone depletion, human health/toxicity and land use degradation).
Not only have concerns broadened beyond that of energy consumption, but the resolution and availability of data on the flows of materials and chemicals within the environment have improved, as has the ability to describe relationships of causality and impact. While embodied energy can be used to understand aspects of manufacturing efficiency and help to evaluate inherent differences between materials and their modes of production, reliance on embodied energy as the sole sustainability metric for the evaluation of materials, architectural products and whole buildings is potentially limiting.
New calculation methodologies and databases, such as multi-impact life cycle assessments, have increased in resolution and offer a more complete understanding of the environmental impact of construction across a range of impact categories. It is also pertinent to observe that the aluminium industry was an early adopter of the use of LCA, as recorded by Elizabeth Trenton.
To illustrate the limitations of focusing on embodied energy, consider the following example. A construction material whose manufacturing relies upon hydroelectric power is not necessarily as impactful as another product whose manufacturing relies upon the same amount of electricity or heat produced by a coal plant, although both products’ embodied energy would be expressed identically.
Hydroelectric power is a renewable energy resource, whereas fossil fuels are a non-renewable resource whose extraction and combustion have clear and definable environmental impacts. Conducting environmental assessments from a potential impact perspective helps to clarify and reveal variations between energy mix and manufacturing processes across space and time. In terms of environmental impacts, not all megajoules are equal. Indeed, the aluminium industry has long accounted for regional energy blends in its own life cycle inventories and impact assessments. At a time marked by concerns over climate change and human health, these concerns are far better tracked through measures of impact, not just inventories.
The author is an architect based in the United Kingdom who can be contacted through his firm’s website www.s4aa.co.uk.
Other information sources and reference documents in this excerpt:
“Recycling is the primary energy efficiency technology for aluminum and steel manufacturing,” United States Energy Information Administration, www.eia.gov/todayinenergy/detail.cfm?id=16211#tabs_SpotPriceSlider-2
European Aluminium Association, “European Aluminium Industry’s Sustainability Roadmap to 2025,” www.alueurope.eu/#3rdPage
European Aluminium Association, “Environmental Profile Report for the European Aluminium Industry,” www.alueurope.eu/wp-content/uploads/2011/10/Environmental-Profile-Report-for-the-European-Aluminium-Industry-April-2013.pdf
Preservation Green Lab for the National Trust for Historic Preservation, “The Greenest Building: Quantifying the Value of Building Reuse,” www.preservationnation.org/information-center/sustainable-communities/green-lab/lca/The_Greenest_Building_lowres.pdf
Advisory Council on Historic Preservation, “Assessing the Energy Conservation Benefits of Historic Preservation: Methods and Examples,” www.achp.gov/1979%20-%20Energy%20Conserv%20and%20Hist%20Pres.pdf
U. M. J. Boin and J. A. van Houwelingen, “Collection of Aluminium from Buildings in Europe: A Study by Delft University of Technology,” http://recycling.world-aluminium.org/uploads/media/_TUDelftBrochure2004.pdf
Z. Bribian, A. Aranda Usón and S. Scarpellini, “Life cycle assessment in buildings: state-of-the-art and simplified LCA methodology as a complement for building certification,” Building and Environment
R. H. Crawford, “Life Cycle Assessment in the Built Environment”
E. Trenton, “The Application of Two Models of Life Cycle Assessment (LCA) for Transition to the Low-Carbon Economy: A Case Study in the Aluminum Industry”
United States Department of Energy, “2010 Building Energy Data Book,” U.S. Department of Energy, http://buildingsdatabook.eren.doe.gov/
J. Marriott, H. S. Matthews and C. T. Hendrickson, “Impact of power generation mix on life cycle assessment and carbon footprint greenhouse gas results,” Journal of Industrial Ecology
International Aluminium Institute, “Life Cycle Assessment of Aluminium: Inventory Data for the Primary Aluminium Industry,” www.world-aluminium.org/media/filer_public/2013/10/17/2010_life_cycle_inventory_report.pdf
Latest from Recycling Today
- Southwire joins Vinyl Sustainability Council
- Panasonic, Sumitimo cooperate on battery materials recycling in Japan
- Open End Auto Tie Balers in stock, ready to ship
- Reconomy names new chief financial officer
- ICIS says rPET incentives remain weak
- New Jersey officials award $16.2M in annual recycling, waste reduction grants
- Linder Industrial Machinery announces leadership changes
- First phase of EPR scheme launching in Alberta