
Over the past three years, the U.S. has made aggressive commitments and investments to reduce greenhouse gas (GHG) emissions that contribute to climate change. One way to reduce GHG emissions and the rate of climate change is by lowering fossil fuel use within the transportation sector.
According to the U.S. Environmental Protection Agency (EPA), the transportation sector is responsible for 15 percent of global GHG emissions, and 95 percent of the world’s transportation energy comes from petroleum-based fuels. In the U.S., according to an article published by the MIT Energy Initiative, passenger vehicles generate about 16 percent of total anthropogenic GHG emissions and consume about 40 percent of the total petroleum used in the U.S. Therefore, policymakers have taken concrete steps to invest in and mandate the development and adoption of battery electric vehicles (BEVs) powered by vehicle traction batteries.
Examples of the aggressive commitments to reduce GHG emissions strongly suggest that BEVs will proliferate rapidly on U.S. roadways in the coming years.
In 2020, California Gov. Gavin Newsom signed an executive order requiring 100 percent of in-state new passenger car and truck sales to be zero-emission by 2035. President Joe Biden signed a similar executive order in 2021 that set the goal that 50 percent of new passenger cars and light trucks sold in 2030 be zero-emission vehicles. These commitments are causing an unprecedented transition away from internal combustion engine (ICE) vehicles to BEVs. Consequently, demand for vehicle traction batteries, battery components and critical materials used in making batteries has increased dramatically.
While the total number of BEVs represents a small overall percentage of the entire passenger vehicle population in the U.S., the number of vehicles containing vehicle traction batteries is growing exponentially and making up larger percentages of the total vehicle population in the U.S. According to the Department of Energy (DOE), 283.51 million light-duty vehicles were registered in the U.S. in 2022. Of this population, 2.44 million vehicles were fully electric (EV), 1.01 million were plug-in-hybrid electric (PHEV) and 6.29 million were hybrid electric (HEV). This is a significant change from 2020’s 1.02 million EVs, 594,400 PHEVs and 4.81 million HEVs.
The transition from ICE vehicles to BEVs could positively transform the U.S. transportation sector by drastically reducing reliance on fossil fuels and associated GHG emissions. However, a successful transition to BEVs will demand that the existing benefits of ICE vehicles be carried over and built upon by BEVs.
ICE vehicles have been successful in providing access to transportation, which positively correlates with an increase in opportunities and economic outcomes, according to the Urban Institute. Over the past several decades, American households have had unprecedented access to motor vehicles because they are affordable to finance, insure and repair. Furthermore, vehicles and their components are durable products that serve multiple owners over long service lives with predictable reliability and value.
While vehicle owners throughout the vehicle value chain can predict the value of used ICE vehicles, one of the biggest challenges facing mainstream BEV adoption is that owners lack a clear understanding as to the quality, performance and remaining service life of a traction battery—the costliest component of the BEV in some cases, according to Interact Analysis. A thriving and robust BEV industry from the sale of a new car until its traction battery is reused and recycled is essential for mainstream and more equitable adoption of sustainable transportation. Proper testing and evaluation of traction batteries will be essential for a successful transition to BEVs, which will help ensure easy and affordable access to passenger vehicles.
The life cycle of an ICE vehicle

With ICE vehicles, an economically viable product life cycle has developed that prioritizes affordability, encourages maximizing existing resources and enables recyclability.
An ICE vehicle’s life cycle is as follows: 1) manufactured by an original equipment manufacturer (OEM); 2) sold as a new car; 3) maintained by the original owner or sold as a used vehicle; 4) reached its end-of-life (EOL) because of a wreck or it is no longer functioning and uneconomical to repair; 5) acquired by an automotive recycler/dismantling facility to be dismantled to reuse repair parts; and 6) crushed and shredded to reclaim recyclables.
Approximately 10 million to 15 million vehicles reach their EOL annually and enter U.S. reuse and recycling infrastructure, according to estimates from the Automotive Recyclers Association (ARA).
Every year, the ARA estimates approximately 10,000 automotive recycling/dismantling facilities throughout the U.S. process millions of EOL vehicles and determine what makes the most economic sense for each vehicle and their components using the principles of highest and best use. A highest and best-use analysis works by identifying the maximum value for each vehicle component, which also often is correlated with the best environmental outcome.
Automotive recyclers/dismantlers have played a critical role in creating a recycled original equipment motor vehicle replacement parts market, a remanufactured vehicle parts market and a recycled materials market. By finding value and promoting reuse, remanufacturing and recycling of all components on EOL vehicles, automotive recyclers/dismantlers have built a circular economy for ICE vehicles that also increases access to transportation by increasing competition in the replacement parts market.
In the case of the U.S. vehicle reuse and recycling market, there is an economic incentive to responsibly and safely process EOL ICE vehicles for reuse and recycling. According to Warrendale, Pennsylvania-based SAE International, 84 percent of vehicles by weight have their components reused, remanufactured and recycled. It is critically important that the success of the existing EOL ICE reuse and recycling market be maintained and replicated moving forward.
An automotive recycler/dismantler processes an EOL vehicle to maximize its highest and best use. The remainder of the vehicle is sent to a scrap processing facility to recover recyclable metals for use in new manufacturing or industrial applications. According to SAE, 95 percent of all vehicles removed from service in the U.S. enter North American recycling infrastructure. The U.S. Geological Survey found that 15 million tons of steel are recycled from automobiles annually. Recycled steel is so important to U.S. manufacturing and infrastructure that an average 93 percent of structural steel produced in the U.S. contains recycled steel, according to the American Institute of Steel Construction.
Besides serving as a primary source for recycled steel, automotive recyclers are a primary source for recyclable aluminum. According to the Worcester Polytechnic Institute, the overall recycling rate for automotive aluminum is 91 percent, and the Aluminum Association finds that 80 percent of U.S. aluminum production comes from recycled aluminum.
Automotive recyclers/dismantlers have a financial necessity to maximize recovery of each component on the vehicle by putting it to its highest and best use. The financial return achieved by an automotive recycler/dismantler from an EOL vehicle determines how much the automotive recycler/dismantler can pay when acquiring a vehicle. Unless an automotive recycler/dismantler can achieve a high recovery rate, it will be unable to acquire vehicles in quantity when bidding against other market segments, such as vehicle rebuilders or vehicle exporters. In the case of BEV vehicles, the ability to acquire vehicles when competing with other industry segments is dependent on whether an automotive recycler/dismantler can put a traction battery to its highest and best use.
BEVs and the highest and best-use hierarchy
The highest and best use hierarchy, which represents the maximization of existing resources in the most economically and environmentally beneficial manner, is as follows: 1) reused as originally intended and unmodified; 2) repaired or reconditioned for original reuse; 3) repurposed for secondary applications; 4) recovered for raw materials for manufacturing; and 5) disposal.
Highest and best use has allowed the U.S. to establish a successful and economically viable framework for managing EOL vehicles. Worcester Polytechnic Institute also found that automotive recyclers/dismantlers help drive a circular economy in auto manufacturing and that the automotive recycling/dismantling industry has a negative carbon footprint.
Governments and industry have made a concerted effort to increase recycling capacity to deal with the impending tsunami of retiring BEV batteries and establish domestic supply chains of battery materials. However, recycling is not a silver bullet for dealing with BEV batteries that have served their initial purpose. Aside from the huge investments required to build up the necessary battery recycling infrastructure, major challenges lie ahead for the battery recycling industry, including transportation and safety, battery pack dismantling and the applicability of recycling technologies to the various battery chemistries.
For instance, the efficiency and profitability of recycling processes are affected by the chemistry of the cathode: lithium iron phosphate (LFP) batteries can cost up to a few dollars per pound to recycle, while nickel-rich chemistries are closer to net positive or breaking even with the resale of raw materials and black mass, according to Argus Media and research by Linda Gaines, et al.
Research by J. Dunn, et al., promotes a battery material use hierarchy, where repurposing and reusing a retired EV battery before recycling are favorable to a straight-to-recycling approach from a life cycle analysis (LCA) perspective. Findings from an LCA by the Energy and Efficiency Institute at University of California, Davis, show that traction battery reuse and repurposing prior to recycling reduces environmental impacts compared with the direct-to-recycling pathway, yielding about 25 percent of the CO2e emissions on a per 1 kWh unit.
The Battery Material Use Hierarchy, produced by ARA and Argonne National Laboratory, emphasizes the need for highest and best use for batteries after they have served their initial purpose. A vehicle traction battery that has reached its end of first life (EOFL) that is in good health can and should be reused in a vehicle. A battery can be remanufactured and put back in a vehicle under a limited warranty, while a medium-health battery may be well-suited for a stationary energy storage system (ESS) application where the environment is less stressful than mobility applications. Use cases encompass utility-scale energy storage, behind-the-meter commercial and industrial storage, residential applications, as well as portable and off-grid battery systems. Each use case is unique and can be optimized by using different battery types of varying ages.

A battery in poor health would be sold for a lower price, or recycled, the lowest preference on the hierarchy.
Following the Battery Material Use Hierarchy ensures automotive recyclers/dismantlers maximize existing resources, provide affordable vehicle repair parts and create a sustainable and circular economy as the transportation industry electrifies.
BEV traction batteries age over time and use, but it’s difficult to assess the health of a battery with today’s commercially available technologies. Unlike an ICE vehicle, where the number of miles on a vehicle’s odometer serves as a good metric for the vehicle’s value, an odometer reading does not provide satisfactory details as to the condition of a BEV or the battery pack. Battery life and condition can change because of temperature, humidity, use and charging conditions—mileage alone cannot adequately assess the condition of a traction battery.
Battery types, chemistries and configurations
Before diving into the intricacies of EOL, it is important to understand one of the highest-value components of a BEV: the high-voltage traction battery.
Different BEVs have different battery configurations, sizes and chemistries. In addition, each battery type will have varying electrical, mechanical and software interfaces that must be accounted for. For instance, a Tesla Model 3 could have either a lithium iron phosphate (LFP) battery pack or a nickel cobalt aluminum (NCA) battery pack, depending on the model year and trim level. A 2011 Nissan LEAF had a 24 kWh lithium manganese oxide (LMO) battery pack, a 2013 Nissan LEAF had a 30 kWh LMO battery pack, while a 2017 Nissan LEAF had a 42 kWh or a 60 kWh nickel manganese cobalt (NMC) battery pack. A Nissan LEAF battery pack is configured from battery modules ranging from 8V nominal to 22V nominal, depending on the vehicle generation. Meanwhile, older Tesla Model 3 battery packs have four 85V to 95V battery modules, and newer generation battery packs, some of which have cell-to-pack design, might not have individual battery modules.
All these aforementioned BEV models use batteries with a “400V” battery pack architecture, but a Hyundai Ioniq BEV uses an “800V” battery pack architecture. In addition, each of these battery systems has its own battery management system (BMS) and interfacing software, much of which is proprietary to the OEM.
BEV battery systems designs have no standardization. Full EVs have been on the market for less than 15 years, but global EV sales didn’t hit 1 million until 2017, according to the International Energy Agency, Paris. With continuous improvements in battery technology and manufacturing capabilities, and with growing geopolitical tensions affecting supply chains, OEMs’ approach to battery design will further diverge before they begin to standardize. This has repercussions downstream in the BEV aftermarket and EOL value chains that affect how and whether highest and best use can be applied to BEVs. For example, as current BEV batteries age and OEMs no longer manufacture or support specific battery types, battery reuse will be needed to support aging vehicles in addition to demand for repurposing of batteries for ESS.
Gaps in battery state-of-health evaluation
Some OEMs display some indication of battery state of health (SOH), but this is known to be unreliable and generally less accurate the older the battery, according to research from M. Bercibar, et al. These health predictions are built on lab-based models and not a result of a real test on the battery. Additionally, historical data from vehicles often is unavailable and unreliable.
Many factors affect battery degradation. In lithium-ion batteries, chemical changes play a significant role, including lithium plating, where solid lithium forms on the anode, and solid-electrolyte interphase (SEI) growth, which hinders ion movement. Cycling-related degradation occurs from repeated charge/discharge cycles, leading to chemical and structural changes in the electrodes. Extreme heat and cold, fast charging and lack of “rest” can cause a battery to degrade faster, leading to premature battery replacement. The average state of charge (SOC) of a battery also influences degradation, where extreme overcharge and overdischarge states cause stress on electrodes. Calendar aging, which is natural degradation over time, and dendrite formation, microscopic needle-like growth on electrodes, are additional factors. Mechanical stress, such as bending or compression, and chemical reactions also affect degradation, according to the Journal of the Electrochemical Society.
Determining the overall health of a battery is challenging because of many factors, including limited availability or use of advanced diagnostic tools, infrequency of testing and the absence of real-time monitoring systems to detect sudden changes during the battery’s operation, according to research from M. Ge et al. Additionally, inaccurate data interpretation, insufficient historical data on the battery’s past performance and incomplete consideration of actual environmental factors can contribute to deficiencies in evaluation.
Addressing these issues involves implementing comprehensive evaluation processes, using advanced diagnostic tools, ensuring regular monitoring and incorporating relevant environmental and usage considerations. Standardized guidelines and effective communication of results are crucial for closing gaps in battery SOH evaluations and enhancing the overall effectiveness of the assessment. Unfortunately, existing solutions to this challenge are not scalable to the larger industry.

Limitations in battery testing
The industry standard in battery health testing is cycling, which requires completely charging and discharging a battery. It can take more than 10 hours to test a single battery using this approach. For example, Tesla allows its customers to test the health of the battery in their vehicles, but the operation requires the vehicle to be plugged into a Level 2 charger for up to 24 hours and to have the vehicle below 50 percent charge. Most consumers don’t have that level of patience, and used car dealers or repair shops don’t have the capability to perform this procedure beyond a one-off test.
Sponsored Content
Labor that Works
With 25 years of experience, Leadpoint delivers cost-effective workforce solutions tailored to your needs. We handle the recruiting, hiring, training, and onboarding to deliver stable, productive, and safety-focused teams. Our commitment to safety and quality ensures peace of mind with a reliable workforce that helps you achieve your goals.
Sponsored Content
Labor that Works
With 25 years of experience, Leadpoint delivers cost-effective workforce solutions tailored to your needs. We handle the recruiting, hiring, training, and onboarding to deliver stable, productive, and safety-focused teams. Our commitment to safety and quality ensures peace of mind with a reliable workforce that helps you achieve your goals.
To perform cycle testing at scale, capital resources and infrastructure must be in place. A battery test facility needs sufficient space to receive, store and test all the batteries at any given time. The right system is necessary to create a unique ID for each battery, ideally tying back to its original application and any historical usage data, and an inventory system is needed to track and locate batteries. High-voltage and high-power test equipment, and the staff capable of operating it, need to be installed on-site. Training in safe battery handling is required for many, if not all, staff members, with some needing hazmat certification.
The inefficiency and cost of this type of operation make these test methods challenging for the roughly 10,000 automotive recycling/dismantling facilities throughout the U.S., creating a major challenge when putting a vehicle traction battery to its highest and best use.
Depending on the intended use, additional requirements might be needed to meet certifications and standards. For instance, UL 1974 is the “Standard for Evaluation for Repurposed Cells for Stationary Applications.” This standard addresses the evaluation and testing of repurposed cells, which refers to batteries that were originally used in one application, such as EVs, and then repurposed for stationary energy storage applications. The goal of UL 1974 is to provide safety and performance requirements for these repurposed cells to ensure their suitability for stationary applications. Unfortunately, acquiring the proper certification to UL 1974 is costly and complex. Additionally, each battery manufacturer and OEM could have their own battery test protocol, which further complicates the picture.
Regulations around the collection, storage and transportation of disposed batteries also are complex. Lithium-ion batteries are considered Class 9 hazardous waste, which means specific rules dictate the packaging and transport of battery feedstock. The Environmental Protection Agency (EPA) also classifies disposed batteries as universal waste, which prohibits landfilling of batteries and the storage of disposed lithium batteries for more than one year. Following, the highest and best-use practices for battery end-of-life management can be very expensive without rapid and accurate battery testing.
Rapid battery testing: A better way forward

ReJoule is an advanced battery diagnostic company with the mission of creating a circular BEV battery economy by enabling battery reuse, remanufacturing and repurposing. It has pioneered an advanced battery test technique to accurately, quickly and reliably estimate a battery’s SOH. The diagnostic technology employs electrochemical impedance spectroscopy (EIS) to estimate the degradation of a battery through its complex impedance. This enables a test time reduction from hours to less than 5 minutes. ReJoule’s hardware product is coupled with machine learning algorithms to correlate a battery’s complex impedance to its SOH.
ReJoule’s technology consistently has achieved more than 98 percent accuracy in its SOH assessment for multiple battery types of different chemistries and can test at the cell, module or pack level.
ReJoule also has developed a version of its diagnostic technology that can plug into the DC fast charging port of an EV to provide rapid and accurate testing on traction batteries that are in vehicles.
This family of diagnostic products not only speeds up the testing process but also can transform battery management strategies, paving the path for proactive measures like predictive maintenance, assessing residual value and forecasting future EOFL traction battery feedstock. By quickly identifying the core issue of degradation, more vehicles can be tested, which reduces the overall cost.
Once the battery’s SOH is determined, charts like the one pictured can assist in quickly directing batteries to the highest value use case. This can transform today’s used BEV market, make it easier to buy insurance for BEVs, improve repairability and repurposability of high-voltage traction batteries and reduce overall emissions by improving the economics of reuse, remanufacturing and repurposing.
ReJoule has demonstrated the effectiveness of its technology on nickel manganese cobalt (NMC) cells and modules and various lithium iron phosphate (LFP) cells and modules and on cylindrical, pouch and prismatic constructions.
As of the beginning of 2024, ReJoule has tested more than 5 MW of retired EV batteries on three continents, with the bulk of that testing done in 2023 alone.
Putting batteries to their highest and best use
Professional automotive recyclers/ dismantlers are the largest collective owners of EOL vehicles and the largest generators of source material for scrap metal recyclers. As the main recipient of EOL vehicles, they also are the largest collective owners of EOFL lead acid, nickel metal hydride and lithium-ion vehicle traction batteries.
ARA and its member companies are taking the lead in this area by actively advancing the national imperative of highest and best use of BEV traction batteries. Through its strategic relationship with the National Salvage Vehicle Reporting Program (NSVRP), ARA and its certified high-voltage vehicle trained members are participating in the NSVRP battery registry program. This program is designed to help ensure that BEV traction batteries that have served their initial purpose are directed to their highest and best use whenever possible.
Get curated news on YOUR industry.
Enter your email to receive our newsletters.
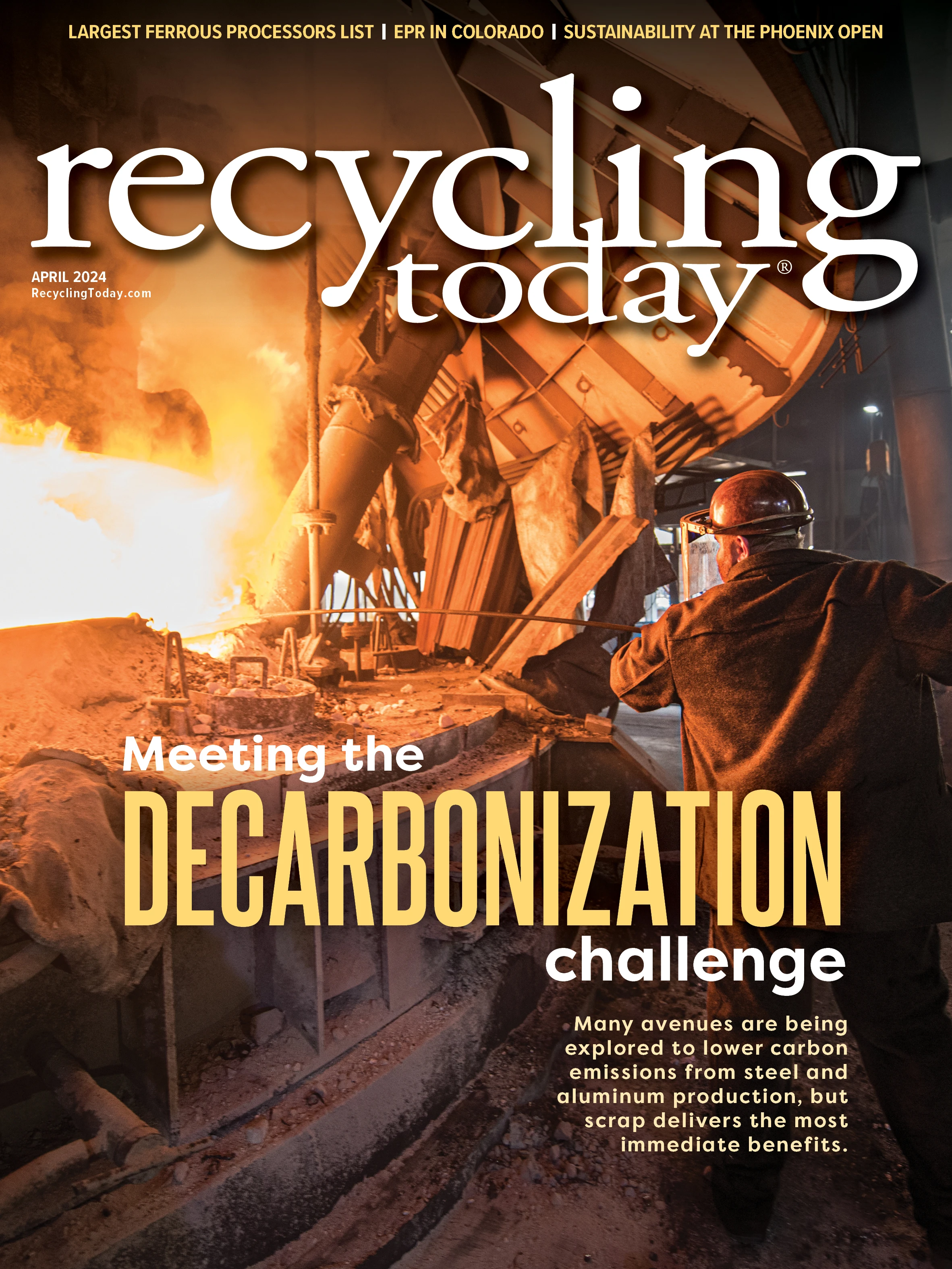
Explore the April 2024 Issue
Check out more from this issue and find your next story to read.
Latest from Recycling Today
- Athens Services terminates contract with San Marino, California
- Partners develop specialty response vehicles for LIB fires
- Sonoco cites OCC shortage for price hike in Europe
- British Steel mill’s future up in the air
- Tomra applies GAINnext AI technology to upgrade wrought aluminum scrap
- Redwood Materials partners with Isuzu Commercial Truck
- The push for more supply
- ReMA PSI Chapter adds 7 members